New study reveals surprising behavior of iron under extreme conditions
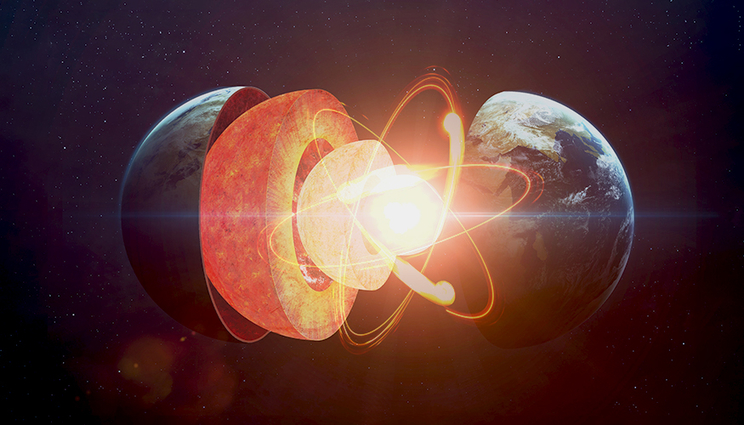
Lawrence Livermore National Laboratory researchers applied nanosecond laser shock compression to iron at pressures up to 275 gigapascals — more than 2 million times atmospheric pressure — and used in situ picosecond x-ray diffraction to study the structure of the iron under these extreme conditions. Researchers said the resulting data improves understanding of materials science and provides insights into the internal dynamics of the Earth’s core, as well as the cores of other terrestrial exoplanets. Adobe stock image.
Iron is one of the world’s most abundant elements and a primary component of the Earth's core. Understanding the behavior of iron under extreme conditions, such as ultra-high pressures and temperatures, has implications for the science of geology and the Earth’s evolution.
In a study conducted by a team led by Lawrence Livermore National Laboratory. researchers combined lasers and X-ray diffraction methods to examine how different crystal structures of iron are related to each other and what happens when it melts at ultrahigh pressures and temperatures. The paper was published in the journal Physical Review B.
Using the Dynamic Compression Sector beamline at Argonne National Laboratory, researchers applied nanosecond laser shock compression to iron at pressures up to 275 gigapascals (GPa) — more than 2 million times atmospheric pressure — and used in situ picosecond X-ray diffraction to study the structure of the iron under these extreme conditions. Authors said the ability to gather this novel data on iron provides insights into materials science and the internal dynamics of Earth and other terrestrial exoplanets.
“The measurements we have made for this work are a result of the amazing contribution of hundreds of scientists at LLNL and elsewhere,” said lead author Saransh Soderlind. “The experiments reported in the paper are becoming more and more routine on many platforms; however, the timescale of the experiments — over a mere few nanoseconds —and the scale of the collaboration required to perform a single experiment boggles my mind. It is surprising to me that some of these experiments have worked so well that we have started making measurements, which finally let us unravel some deep questions that we've had about the formation of Earth.”
Earth’s core consists primarily of solid iron with some lighter elemental impurities, surrounded by a layer of mostly liquid iron. As the Earth cools, the core grows over time through recrystallization at the liquid-solid boundary between the outer and inner core. The process of recrystallization releases heat which contributes to the convection currents in the outer fluid core. This convective flow of liquid iron has long been thought to power the Earth's geodynamo — the process responsible for generating the Earth's magnetic field.
In the study, the team found that the contribution to the geodynamo energy budget from the heat release from recrystallization may be significantly smaller than previously thought. The findings suggest that outer core convection currents are driven more prominently from alternative sources, such as lower-mantle radiogenic heat generation and the buoyancy of light elements excluded from the solid during the recrystallization process.
“There’s very little data on the behavior of iron at these extreme pressures and temperatures, so our contribution is providing the experimental measurements that complement years of theoretical work and will help constrain the amount of heat released from iron as it melts,” said the study’s co-principal investigator and LLNL physicist Ray Smith. “There are many contributors that people have come up with for convection currents in the outer core that would power the geodynamo. Theory would say one thing, based on their understanding of iron, but the experiments in our case are presenting a different view — that the process of the heat released from recrystallization is less than the state-of-the-art theoretical calculations.”
Smith said the study is the first to gather liquid diffraction data on laser shock-compressed iron. Through their experiments, the team determined the highest-pressure structure and density of liquid iron, indicating that iron melts completely by 258 GPa and behaves as a “simple liquid” under extreme conditions. The melt pressures for the nanosecond shock experiments were consistent with gas gun shock experiments that last for microseconds, indicating that the melt transition occurs rapidly, researchers concluded.
Additionally, the team demonstrated the efficacy of the picosecond X-ray diffraction platform — also a first for this type of research — in investigating the liquid phase of iron under extreme conditions, from the beginning of the melt to the completion of melt. Using diffraction methods, the team aimed to pinpoint the precise mechanisms behind iron’s phase transformations, performing texture analysis as the atoms in the iron reorientated themselves from an ambient crystal structure to the high-pressure phase.
The researchers found that when iron is subjected to high pressures and temperatures, it undergoes a phase transition from its usual body-centered cubic (bcc) structure to a hexagonal close-packed (hcp) crystal structure — and as pressure increases, the hexagonal structure eventually melts into a liquid. The transition from bcc to hcp is “not sharp,” Soderlind said, and instead the two structures coexist over a range of pressures.
“Materials subjected to elevated pressures can respond by phase transforming to a lower-energy crystal structure with profound changes in mechanical and transport properties,” Soderlind said. “Scientists have studied these transitions through theory and experiments for over a century. The precise way the atomic motion occurs to bring about such a change is scientifically intriguing and provides stringent tests on our best models.”
Soderlind said characterizing iron’s liquid structure is invaluable for comparing to predictions from state-of-the-art theoretical models, such as quantum molecular dynamics simulations. Although researchers could not determine the exact mechanism behind the phase transition in this study, the work provided crucial details about the coexistence of different iron phases and latent heat of fusion at extreme pressures, as well as essential data for future identification, Soderlind said.
“In this paper, we tried to measure this precise mechanism using X-ray diffraction. While we could rule out many proposed mechanisms, we could only reduce it to three choices, all consistent with the data,” Soderlind said. “This was a significant step forward. The experiment also gave us clues to what we would need in future experiments to identify this mechanism uniquely.”
Since the composition of the core-mantle boundary is not pure iron, and instead includes nickel and other light elements, Soderlind explained, future research could involve studying a range of iron-nickel alloys to better understand the composition of the Earth's core.
The team has conducted a follow-up experiment with one composition of iron-nickel alloy and plans another involving two additional compositions, which should give the team a more accurate measure of the energy contribution due to core solidification, Soderlind said.
Additional co-authors on the paper include Richard Briggs, Martin Gorman, Lorin Benedict, Christine Wu, Sebastien Hamel, Amy Coleman, Federica Coppari, Christopher McGuire, Jon Eggert and Dayne Fratanduono of LLNL. Other contributors were Amalia Fernandez-Pañella of the Gordon and Betty Moore Foundation, and Melissa Sims and June Wicks of Johns Hopkins University.
Contact

[email protected]
(925) 422-5539
Related Links
Structural study of hcp and liquid iron under shock compression up to 275 GPaTags
Lasers and Optical S&TEngineering
Physical and Life Sciences
Physics
Featured Articles
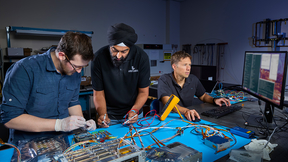
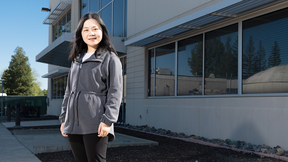
